Tissue engineering is rapidly growing as a therapeutic alternative in regenerative medicine. Functional biomaterials research continues to develop and improve on scaffolding as it’s applied for the engineering of cells and tissue to repair and/or regenerate an organ or tissue. Scaffolds can be defined as, materials that have been engineered to cause desirable cellular interactions to contribute to the formation of new functional tissues for medical purposes. Cells are often ‘seeded’ into these structures capable of supporting three-dimensional tissue formation. Scaffolds utilizing natural polymers have been developed and continue to gain more popularity. These include chitosan, a biopolymer derived from the alkaline deacetylation of chitin. Chitosan is now arguably the most researched biopolymer in recent history, and interest in its applications in tissue engineering continues to build. This interest is primarily due to chitosan’s chemical and biological properties as they inherently enable useful functionalities and versatility as a biomaterial. These biological properties include its biocompatibility, biodegradable, and bioactive, facilitates its use for scaffolding in tissue engineering and for repair and/or regeneration of various tissues including skin, bone, cartilage, nerves, liver, and muscle. In this review, the intrinsic properties, characteristics, and key aspects of chitosan is examined for its scaffolding capabilities in tissue engineering and regenerative medicine.
Introduction
Today, regenerative medicine is one of the most popular scientific fields, where new technology and today’s public health challenges converge.
Regenerative medicine is defined as the association of tissue engineering, stem cells research, gene therapy, and therapeutic cloning, as important strategies for regenerative medicine. Biomaterials research is focusing on the design, development, and improvement of scaffolds as new drug release systems and bioactive molecules for regenerative medicine [1]. All of this has been made possible due to dramatic advances in tissue engineering, especially in the last 10 years.
The objective of tissue engineering is to restore, regenerate, maintain, or improve function in defective tissue or lost tissue due to various disease conditions and injuries. Biologic substitutes are used to induce tissue regeneration. With this, structural scaffolds are often used to replace and/or rebuilding lost or damaged tissue and organs. Tissue engineering is known as the use of isolated cells or cell substitutes, tissue inducers, and cells placed on or in matrices to repair and regenerate tissue [2]. Tissue engineering techniques can be generally classified into 3 groups:
(1) Isolated cell implants or cell substitutes in the body
(2) Tissue inducer substances (such as growth factors), and
(3) Cells placed on or in matrices or substrates that work as a vehicle or scaffold that induces tissue regeneration [3].
Thus, the focus of tissue engineering points to the use of structures used to repair injured tissue or tissue with structural malformations and also reinforce and, in certain cases, organize regenerating tissue that would work as a scaffolding to restore or regenerate the damaged tissue [4].
The follow are important characteristics of chitosan for its use in tissue engineering and regenerative medicine;
- Biocompatibility – Binds to receptors and can be tuned to mitigate rejection
- Absorbability and degradability – Absorbable and can control degradation and resorption rates to be in line with in vitro and in vivo cell/tissue growth. – Allows the fabrication of structures with properties that align to the needs of the receiving tissue to regenerate and repair.
- Nontoxic and non-carcinogenic – Its degradation products cannot cause local or systemic adverse effect on a biological system
- Chemically stable – Chemical modifications not being present in a biological system implant or biodegradable in nontoxic products, at least during the scheduled time to regenerate tissue
- Sufficient surface area – Has chemically adequate surface area for cell access, proliferation and cell differentiation
- Adequate resistance and mechanical properties – Resistance and mechanical properties, superficial characteristics, fatigue time, and weight, inline to the receptor tissue needs
- Proper design, size, and shape of the scaffolding – Allows generation of structures with properties that align to the needs of the receiving tissue to regenerate and repair
Scaffolding
Scaffolding is the use of 2D or 3D porous solid biomaterials, designed to:
(1) Promote cell-biomaterial interactions, cell adhesion, cell proliferation, and extracellular matrix deposits (ECMD)
(2) Facilitate the transport of gases, nutrients, and regulatory factors to enable cell survival, proliferation, and differentiation
(3) Control degradation rates which align with regeneration rates of native tissue
(4) Mitigate unwanted inflammatory responses.[5]
Chitosan used as scaffolds in tissue engineering must possess various functional characteristics (…see Table 1) in order to elicit desired effects, and ultimately result in desirable medical outcomes [23]. Chitosan scaffolding is often used in tissue engineering as it possesses these functional characteristics and thus can be an alternative to the limitations of autograft and allograft tissues [23].
Chitosan is biocompatible for applications in regenerative medicine and tissue engineering. Its a natural biodegradable biomaterial. It’s flexible in terms of chemical manipulation and the ability to break-down into low molecular weight fragments that can be eliminated or resorbed by the human body [24, 25]. Due to this polysaccharide’s bioactive properties, it tends to promote more biological interaction and functional performance with cells compared to synthetic materials.[26].
Chitosan continues to be used in medical devices and scaffolds in tissue engineering more and more [27, 28]. This is due to its multifaceted functional characteristics such as:
Chemical composition, Molecular weight, Solubility, Shape and structure, Hydrophilicity/hydrophobicity, Mechanical properties, Biocompatibility, Surface energy, Water absorption capacity, Controllable biodegradability – breakdown and erosion mechanism, high surface-volume ratio, Superior porosity on the surface with a very small pore size
Tensile strength and stretchability, Tissue, cell, and mucosa adhesion, and Versatility in surface chemistry.
The width, depth, and versatility of these functional characteristics of chitosan has attracted a great deal of attention its use for scaffolding in tissue engineering applications in regenerative medicine for repair and replacement of bones, tendons, and ligaments, skin, organs, cells, tissues, etc. [29].
Figure 1: Chemical structure of chitosan
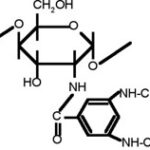
Chitosan characteristics
Chitosan is a linear polysaccharide composed of randomly distributed β-(1→4)-linked D-glucosamine (deacetylated unit) and N-acetyl-D-glucosamine (acetylated unit). (Figure 1), in which glucosamine is the predominant repeating unit in its structure; it is a derivative of the alkaline deacetylation of chitin, and the glucosamine content is named according to the degree of deacetylation (DD). Its molecular weight (Mw) varies depending on the source starting material of the chitin and its processing into chitosan. But in general, commercially available chitosans’ Mw varies between 200 kDa to 1,000 kDa, and with a DD between 75% and 95%. Chitosan has been the most researched and used version of the chitin polymer primarily due to its easily soluble in diluted organic acids, thereby having a greater availability to be used in chemical reactions [27, 28, 31–33].
Chitosan properties are very much affected by the manufacturing process and conditions are produced in, such as the resulting amount of deacetylation. The DD in chitosan is a key feature that determines its physical, chemical, and biological characteristics. The DD is determined by the number of free amino groups in the polymer chain, and this free amino group confers a positive charge to chitosan. The amino group and the hydroxyl group provide functionality, so chitosan turns out to be a highly reactive polysaccharide. The positive charge in chitosan allows for many electrostatic interactions with negatively charged molecules. The processing conditions, as well as the number of functional groups created by deacetylation, allow for the coupling of the groups, which has an impact on the crystallinity of chitosan. In turn, this is associated with the ability of chitosan to be soluble in aqueous acid solutions [34].
Chitosan has many physical and chemical properties conferred by its functional groups (amino NH2 and hydroxyl OH), as well as biological properties from its chemical composition. Solubility, biodegradability, reactivity, and absorption of many of its substrates depend on the amount of protonated amino groups in the polymer chain, and thus in the rate of acetylated or nonacetylated glucosamine [35, 36]. All of these features make it an attractive option for several applications in science such as food/nutrition, medicine, microbiology, immunology, agriculture, and veterinary medicine [37].
Chitosan physiochemical properties
pH Dependence and solubility depends on the distribution of free amino and N-acetyl groups. In diluted acid solutions (pH ≤ 6) the free amino groups are protonated and confer a polycationic behavior and the molecule becomes soluble [4]. Similarly, when the amino groups (pka 6.2–7.0) are completely protonated in acids with a pka less than 6.2, chitosan is rendered soluble, and remains so until a pH ~6.2 is reached. Whereas, when at a higher pH (~> 6.5), the amines in chitosan deprotonate and chitosan becomes insoluble, after which precipitates, such as hydrated gels, are formed. Chitosan is insoluble in water, aqueous solutions, concentrated acids, and common organic solvents. But its easily soluble when stirred into aqueous solutions such as acetic acid, nitric acid, hydrochloric acid, perchloric acid, lactic acid, and phosphoric acid [36, 38, 39].
Degree of Deacetylation (DDA). The degree of deacetylation (DDA) represents the rate of D-glucosamine units to the total amount of N-acetyl-D-glucosamine which makes up the chitosan molecule since this unit is found in the amino group created from the elimination of the acetyl group. A deacetylated chitin (starting over 60 or 70%) is already considered to be chitosan. The DDA is its structural parameter that determines some of its physical and chemical properties, such as solubility limit in acid solutions (pH 2-6), molecular weight, and mechanical properties (elasticity and traction resistance). The deacetylation process of converting chitin into chitosan transforms the acetyl group into a primary amino group, which is more hydrophilic than the preceding molecule. Whereby, the DDA in chitosan increases the water content in samples taken from chitosan, which tends to have an impact on its ability to absorb water, and limits its ability to reach maximum swelling [40].
The DDA also has an impact on chitosan’s biological properties, such as in vitro and in vivo biodegradation. At higher DDA (starting ~>84%), its degradation process is delayed. Highly deacetylated chitosan (over 85%) in an aqueous environment exhibits a low degradation index with degradation occurring only after a few months. Whereas at a lower DDA (~ 65%) degradation occurs much faster. Commercially available chitosans generally have a DDA between 60 and 98%. The DDA of chitosan has an impact on many biological properties of chitosan, such as healing capacity, increase in osteogenesis, and a breakdown process by lysozymes in biological systems [41, 42].
The DDA also plays a key role in cell adhesion and proliferation but does not change the cytocompatibility of chitosan. “In vitro” studies have shown that the lower the DDA in chitosan, the lower the cell adhesion in biofilms. It has also been found that keratinocytes attached to the chitosan biofilm may alter their adhesion and cell growth depending on their DDA, but proliferation is not promoted. Thus, DDA affects the growth of cells in the same way as cell adhesion.
Molecular Weight. Depending on where and how the preparation procedure is done, the molecular weight may change from ~200 to over 1000 kD [4]; viscosity and molecular weight are inversely proportional to the degree of acetylation. Therefore, the greater the molecular weight, the more viscous chitosan membranes tend to be, thus allowing the control of membrane fluidity. This is an important feature in membrane-tissue interaction. Due to its high molecular weight and its lineal nonbranched structure, chitosan is a strong viscosity-building agent in acid mediums and behaves as a pseudoplastic material, where viscosity depends on agitation [43].
Chitosan’s molecular weight also has an indirect effect on swelling and permeability. That is, molecular weight is inversely proportional to the swelling capacity and/or hydration of chitosan membranes. The higher the molecular weight and the DDA, the more membrane swelling, and permeability is reduced [44].
A direct association between chitosan’s molecular weight and DDA is also seen. They both have a direct effect on the biodegradation process of chitosan. The higher the molecular weight the more delayed the degradation process becomes in “in vitro” as well as “in vivo” systems [45]. After oral administration in rodents, decreases in molecular weight led to greater absorption into the liver, kidney, spleen, thymus, heart, and lung [44, 46].
Porosity. The porosity of chitosan used for scaffolding in tissue engineering acts as a platform that provides physical support and to guide differentiation and proliferation of cells for tissue growth “in vivo” and “in vitro.” The porous scaffolding must be similar to the extracellular matrix of the receiving tissue to allow organization of cell growth and neovascularization. Chitosan scaffolding has been extensively investigated in tissue engineering for this very reason, and specifically for soft tissue replacement due to its ability to retain water [47].
Chitosan’s high porosity is necessary for scaffolding in tissue engineering if it’s to be used as structural support and promote cell proliferation. It should have sufficient surface area for live cells to grow, and the precise pore size so facilitate growing cells’ penetration and their proliferation. The desired result is to have a highly interconnected pore structure that allows the transport of nutrients in and between cells for their growth (Figure 2) [48].
Several methods are used to prepare 3D porous scaffolds, among which we find that of thermally induced phase separation (TIPS) in which temperature is reduced to freezing conditions to induce phase separation in a degradable homogenous polymer. Since chitosan is a polymer, it can produce porous membranes to be used as scaffolds [49].
Porous scaffoldings resulting from the TIPS method can synthesize different structures that contain different pore shapes and sizes ranging from 1 to 250 ?m, and that vary according to temperature and water content. For example, the lower the temperature and the greater the water content, the smaller the pore size. Porosity in chitosan membranes has a direct effect on its surface size. Hydrated porous chitosan membranes tend to have at least 2x more surface size and volume compared to nonporous chitosan membranes. But their elasticity and resistance to traction are 10x times less than nonporous membranes [50]. Chitosan’s porous membrane structure can be stabilized by adding glutaraldehyde, polyethyleneglycol, heparin, or collagen. This allows the structure to be more resistant and maintain its elasticity. Despite this caution should be taken since these compounds can cause chitosan to turn insoluble in acid solutions, and result in pore forms to be closed structures [51, 52].
Ideally for scaffolding, chitosan should have an 80 to 90% porosity with a pore size of 50 to 250 ?m. Its pores should be an interconnected mesh to provide physical support to cells, guide cell proliferation and differentiation, and facilitate neovascularization. For example, pore sizes recommended for skin scaffolding should be >160?m, varying between 15–100?m and 100-200 ?m. , with a desired 90% porosity to provide the necessary space and surface to grow cells creating a scaffold for implantation which would result in the regeneration of damaged tissue [53].
Water Absorption Capacity. When placed in an aqueous media, chitosan membranes can swell and retain a given water volume absorbed from the medium in their 3-dimensional network. In order to be used for tissue engineering purposes, they should absorb bodily fluids, promote cell transference, and allow for adequate distribution of nutrients, metabolites, and growth factors, through extracellular media [47].
Mechanical Properties. In the past, chitosan’s mechanical properties had the disadvantage of producing membranes that were very stiff and brittle. That is, they had low mechanical resistance [23]. But now, crosslinking agents, with at least two functional reactive groups that allow for bridging between polymeric chains, are being used. Crosslinkers such as, formaldehyde, epoxides reacting with polyethyleneglycol (PEG), dialdehydes (glutaraldehyde and glyoxal), starch, etc. optimize resistance and elasticity of the chitosan materials [54]. In chitosan cross-linked hydrogels, polymeric chains are bound by the crosslinking agent, which can build a 3D network. The hydrogels’ functionalities are mainly dependent on the density or degree of crosslinking; namely the ratio of moles in the crosslinking agent with the moles in the repeat units of the chitosan. One of the many reactions seen with crosslinked chitosan, is the aldehyde-amine reaction with polyethylene glycol. This has resulted in improvements in mechanical properties, primarily due to PEG’s hydrophilic characteristics. Studies have been that chitosan- DiepoxyPEG (Diepoxy-PEG) crosslinking resulted in marked improvement in the mechanical properties (resistance and elasticity) of the compound [54, 55].
Biological Properties. Chitosan’s many beneficial biomedical properties, such as biocompatibility, biodegradability, and no toxicity is closely related to its solubility, and therefore its molecular weight and DD [56].
Biodegradability. The process of biodegradation of chitosan can occur through various media both physical (thermal degradation) and/or chemical (enzymatic degradation). Its rate of degradation is inversely proportional to the degree of crystallinity, and thus it’s DDA. Therefore, chitosan’s rate of degradation rate can be tuned by altering its DDA [41, 57].
There is a broad range of hydrolytic enzymes responsible for chitosan degradation in “in vivo systems”. But, lysozyme is the primary enzyme. It can be found in lymphoid human and animal tissue and that can be used to naturally degrade chitosan [58, 59]. In the body it leads to the release of amino sugars that can be processed and released by the metabolic system [35].
Some specific enzymes that degrade chitin and chitosan have a clearly identified structure, but their mechanisms of action are still unknown. In mammals, these enzymes seem to be completely absent. However, when chitosan is implanted into the body, it will eventually degrade completely over time. The time and speed of this degradation seems to be dependent on chitosan’s DDA [35].
Through enzymatic hydrolysis mechanisms, chitosan may be easily depolymerized due to susceptibility ? bonds (1–4) mediated by different hydrolases including lysozymes, pectinase, cellulases, hemicellulases, lipases, amylases, and others. Therefore, chitosan has a peculiar vulnerability to enzymes that are different from chitinases. Its degradation products are oligosaccharides or monosaccharides, natural metabolites of glycosaminoglycans or glycoaminoproteins. Lysozyme is an unspecific proteolytic enzyme common in mammals. It can hydrolyze chitosan, but this action is not present when chitosan has a degree of acetylation (DA) below 30% [41].
When chitosan is fully acetylated, it becomes insensitive to this enzyme. Moreover, it seems that at least three consecutive N-acetylated groups are necessary to be recognized by this enzyme. Despite depending on the amine content in chitosan, unspecific enzymes that degrade chitosan are inactivated; such degradation characteristics can be seen in in vivo implants. Many studies have demonstrated that many diverse factors contributing to the biodegradation process of chitosan. But the most impactful are chitosan’s DDA, molecular weight, degree of crystallinity, water content, and the shape and condition of the surface on the material [41].
Biocompatibility. Chitosan is a biocompatible biomaterial. When in contact with the body no recurrent adverse reactions occur. In general, it recognizing and interacts harmoniously with structures and cells of the human body, and vice versa [60]. To date, clinical studies have not reported inflammatory or allergic reactions following implantation, injection, topical application, or ingestion of chitosan in the human body [40]. This is maybe due to that chitosan is made up of GlcN and GlcNac, which are natural components of mammalian tissues [61].
Chitosan is well-established as a biocompatible biomaterial. Toxicity reports have shown that chitosan films’ showed no toxicities using standard “in vivo” safety/toxicity testing [62]. The biocompatibility of chitosan at different DDA. For example, subcutaneous implantation of chitosan in rats demonstrated that the chitosan composite with DDA between 69 and 74% induced a relatively acute inflammatory reaction by rapid biodegradation, with almost complete resorption after 4 weeks of implantation. Composites with chitosan with a high DDA (between 74 to 90%) resulted in a mild inflammatory reaction in tissue degradation rate and a slower rate. This is in agreement with the well-known fact that rapidly biodegradable biomaterials elicit an acute inflammation reaction due to a significantly large production of low-molecular-weight compounds within a short time. Therefore it was determined that films with ≥ 84% DDA showed reaction to softer tissue because these were degraded more slowly [45].
Nontoxicity. Several studies have proven that, so far, clinical tests conducted with chitosan have not reported adverse inflammatory or allergic reactions when used in tissue engineering or as a vehicle in drugs, nor after implantation, injection, or topical application in the human body or for oral application. This property is due to the fact that chitosan is made of GlcN and GlcNAc, natural components of mammalian tissue [41, 42, 63].
Figure 2. Macroscopic images of porous chitosan scaffolds
Chitosan Cytocompatibility
A number of cells have been successfully cultured on 2D and 3D chitosan matrices for cell-based regenerative therapies, e.g., keratinocytes, chondrocytes, osteoblasts, hepatocytes, and Schwann cells [40, 64–68]. Some studies found that the DDA was an important parameter affecting cell adhesion in a number of anchorage-dependent cells, such as keratinocytes, fibroblasts, dorsal root ganglion neurons, and Schwann cells [69–71].
In other studies, the effect of chitosan films’ DDA levels (51-96%) and their effects on osteogenic cells was investigated in porous matrices. These studies revealed a correlation between increases in DDA with cell adhesion. DDA >91% showed a marked improvement in cell adhesion, and osteogenic response [72, 73].
Nonimmunogenic
Chitosan and its oligomers, such as oligosaccharide stimulate macrophage activity increasing nitric oxide, reactive oxygen species, TNF-?, interferon, and IL-1, as well as TGF-?1 and PDGF. However, since there are no proteins and lipids in its structure, it is not possible to develop specific antibodies against it, unless it is coupled with other substances such as albumin. Studies confirm that chitosan is hypoallergenic and only transiently stimulates the immune system. When impregnated into tissue fluids in the body, chitosan ultimately becomes tolerated and metabolized [41, 63, 74].
Antimicrobial and Antifungal
Chitosan inhibits the growth of many types of potentially pathogenic organisms, such as fungi, yeasts, and bacteria. In acid dilute solutions consisting of their positive loads interact with the negatively charged residues of macromolecules on the cell surface of microorganisms, supposedly competing against the Ca+2 for the electronegative sites in the membrane. But it does so without conferring dimensional stability or weakening membrane integrity [75]. Many studies have been conducted examining chitosan’s antimicrobial effects of chitosan on a number of microorganisms, including Candida albicans, Enterobacter cloacae, Enterococcus faecalis, Escherichia coli, Klebsiella pneumoniae, Pseudomonas aeruginosa, Staphylococcus aureus, and Streptococcus pyogenes.
Besides, chitosan’s hemostatic effects, its antimicrobial activity is paramount in the growth of products using chitosan in bandaging materials and wound dressings. These properties, along with its other bioactivities has led to reduced cytotoxicities and enhancement in the healing process of these products. Since chitosan’s antimicrobial effects come directly from the membrane, there is a significantly reduced need for the use of antibacterial substances, frequent bandage changes, or in medical device implants themselves [63].
Conclusion
Regenerative medicine continues to faces challenges as it seeks to induce tissue repair in live tissue. Advances in the use of chitosan scaffolds have led to great excitement in this area as chitosan has shown to have regenerative effects by facilitating cell, tissue, and organ repair. But beyond that, chitosan is being considered an ideal polymer for producing bioactive compounds [131]. This is made possible due to the synergies being seen in their byproducts when conjugated with growth factors and stem cells, either of mesenchymal or neural origin. Future studies should seek to confirm chitosan’s regenerative and bioactive capabilities as a scaffolding tool. Moreover, these studies should seek to translate into real-life tissue repair capabilities in clinical situations.